Electronic Materials Discovery Research
NREL's materials discovery and design work on electronic materials focuses on neuromorphic computing, quantum computing, and thin-film transistors as well as traditional microelectronics.
Materials for Neuromorphic Computing
Processing and storing information already constitute a significant fraction of the global energy demand and are expected to continue to grow rapidly. Neuromorphic computing promises to address this problem by operating similarly to the human brain, which is over 1 million times more energy-efficient than modern computers. Unfortunately, efficiently recreating the complex dynamics of neurons and synapses with inorganic materials is quite difficult. Biological signals can be mimicked by quantum-phase transitions, which carry an inherently low energy burden.
For more information, see Metal Chalcogenides for Neuromorphic Computing: Emerging Materials and Mechanisms, Nanotechnology (2021).
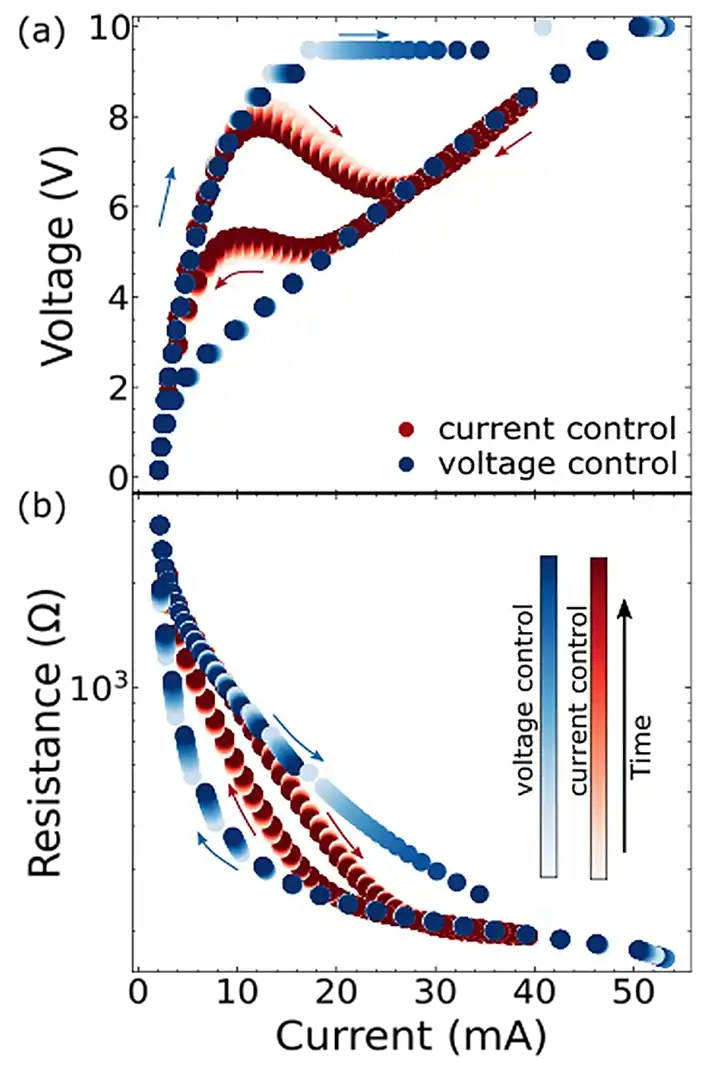
A promising mechanism leveraged for neuromorphic computing is the insulator-metal transition (IMT), a temperature-dependent structural or electronic phase change resulting in a large conductivity change. This robust and repeatable mechanism can enable oscillatory circuits with biologically realistic dynamics similar to that of a neuron. However, current materials operate at temperatures too low or too high for efficient operation.
We seek to leverage a fascinating family of materials, rare earth nickelate distorted perovskites (RNiO3 where R is a rare earth element), to enable efficient and tunable IMT behavior in nanopatterned and biologically realistic neuromorphic spiking circuits. This is accomplished through high-quality epitaxial growth of distorted perovskite heterostructures and nanoimprint lithographically defined structures, aided by simulation program with integrated circuit emphasis (SPICE) circuit models and first-principles materials computation methods.
For more information, see:
Modeling the Impacts of Material Properties on Oscillatory Neuron Behavior, IEEE Transactions on Electron Devices (2024)
Electrically Driven IMT and Volatile Memristor Behavior in NdNiO3 films, Journal of Physics D: Applied Physics (2024)
Synthesis of Tunable SnS-TaS2 Nanoscale Superlattices, Nano Letters (2020).
Materials for Quantum Computing
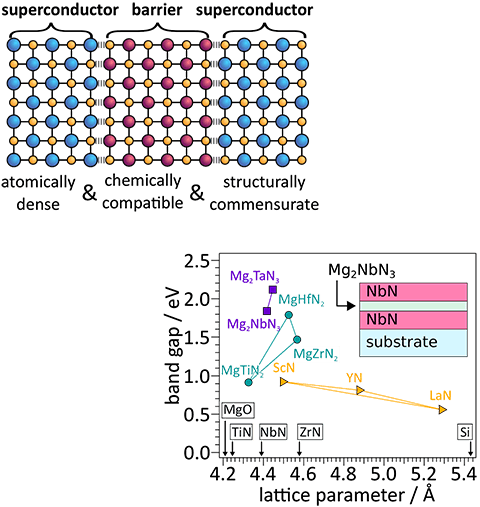
Quantum computers hold potential to solve scientific and energy-related problems intractable for classical hardware and algorithms. One promising technology for quantum computers uses superconducting circuits to act as artificial atoms. Limiting such approaches are materials defects in the constituent circuit elements, which consist of various superconductor, insulator, and interfacial layers. These defects break quantum coherence, which causes quantum computations to be lost before they can be read out.
NREL materials discovery and design researchers are working on low-defect density materials platforms for superconducting quantum circuits. Based on rock-salt nitrides and enabled by the results of our materials design research, we have identified a unique suite of materials such that each element is inherently low-loss, chemically compatible, and structurally commensurate. This work aims to both understand the underlying materials defects that lead to decoherence as well as build epitaxial superconducting quantum circuits from them.
For more information, see:
Epitaxial Growth of Rock Salt MgZrN2 Semiconductors on MgO and GaN, Applied Physics Letters (2020)
Ternary Nitride Semiconductors in the Rocksalt Crystal Structure, Proceedings of the National Academy of Sciences (2019).
Materials for Thin-Film Transistors
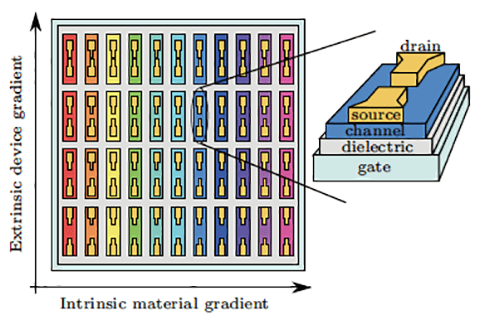
We apply high-throughput experimental methods to thin-film transistors, including combinatorial channel layer growth, device fabrication, and semi-automated characterization. This allows for both extrinsic and intrinsic device gradients to be generated in a thin-film transistors library such as channel thickness, width, or composition.
For more information, see High-Throughput Fabrication and Semi-Automated Characterization of Oxide Thin-Film Transistors, Chinese Physics B (2020).
Projects
NREL's Laboratory Directed Research and Development program has funded electronic materials research projects.
NREL's Laboratory Directed Research and Development (LDRD) program funded this project to explore insulator-metal transitions in rare earth nickelate materials and to leverage this unique physical behavior to create artificial neuron analogs.
NREL's LDRD program funded this project to prepare heterojunctions of structurally commensurate and chemically compatible layers of metal nitride semiconductors and superconductors and then assess the heteroepitaxial trilayers as building blocks for superconducting qubits.
NREL's LDRD program funded this project to explore the synthesis of superlattices of quasi 2-dimensional metal chalcogenides and how superlattice structure modulates the charge density wave transitions of the host materials.
Research Collaborators
Mirzo Mirzokarimov – Device processing
Ian Leahy – Electrical transport
Ella Lachman – Quantum materials and devices (Rigetti Computing)
Dave Pappas – Quantum materials and devices (Rigetti Computing)
Riley Need – Materials characterization (University of California San Diego)
Contacts
Share
Last Updated July 8, 2025